Excerpt from the full article:
Casagli, N., Guzzetti, F., Jaboyedoff, M., Nadim, F., Petley, D., 2017. Hydrological risk: landslides. In: Poljanšek, K., Marín Ferrer, M., De Groeve, T., Clark, I. (Eds.). Science for disaster risk management 2017: knowing better and losing less. EUR 28034 EN, Publications Office of the European Union, Luxembourg, Chapter 3.5, doi: 10.2788/688605
3.5.5 Landslide monitoring and early warning
“These systems [Early Warning Systems: EWSs] require a fine assessment of the socioeconomic impact of landslides, which must be based on accurate landslide mapping, as well as an understanding of their causes. EWSs for landslides are based on the reliable and continual monitoring of relevant indicators (e.g. displacements, rainfall, and groundwater level) that are assumed to be precursors to landslide triggering or reactivation. When the values for these indicators exceed predefined thresholds, alarms are transmitted directly to a chain of people in charge of deciding the level of warning and/or emergency that must be transmitted to the relevant stakeholders, following a predefined process (Figure 3.27). In some cases, warnings can also be automatically transmitted. Usually, one to five alert levels are used (Blikra, 2008; Intrieri et al., 2013): the highest level may lead to emergency warnings to the population, evacuations or the use of sirens and loudspeaker messages in several languages to force people to move to a safer place, as in the case of tsunamis induced by landslides.
An EWS needs to be set up with specific requirements. First, the potential impacts must be defined based on a risk analysis informed by hazard mapping, and including the impact of global changes (Corominas et al., 2014). In addition, the causes and triggers of disasters must be thoroughly analysed and the development of local coping capacities must be included (Dash and Gladwin, 2007).
The number of EWSs dedicated to landslides has greatly increased since the beginning of the 21st century because of the progress made in electronics, communication and computer programs for monitoring and imaging. In addition, the innovations in satellite technologies and ground remote sensing have greatly improved the capacity of remote imaging measurements versus in situ point measurements (Tofani et al., 2013). Implementing an EWS depends on the context, namely (1) the type of landslide (Hungr et al., 2014), (2) the disaster scenarios considered, (3) the degree of awareness of the stakeholders, including populations, and (4) the allocated resources (e.g. budgetary, human). Landslide types determine, first, if the appropriate EWS must be site specific or regional (Intrieri et al., 2013), and second if it is dedicated to identifying triggering conditions and/or to detecting an ongoing event (Sättele et al., 2016). For example, monitoring systems of debris-flow or shallow landslide EWSs are usually based on thresholds of rainfall amount over a period of time. These thresholds are based on rainfall intensity-duration, cumulated event rainfall-duration (Guzzetti et al., 2008), or antecedent precipitation (including snow depth) measures and soil moisture (Baum and Godt, 2010; Jakob et al., 2012). An extended monitoring of those indicators usually makes it possible, therefore, to set regional alarms. Landslide types also constrain the maximum lead time or time of reaction after the alarm trans transmission (Sättele et al., 2016). In some specific cases, debris-flow catchments are equipped with monitoring systems such as ultrasonic and seismic sensors that detect the debris-flow movements (Marchi et al., 2002) and automatically send a warning message to shorten the reaction time as much as possible.
For site-specific systems, displacements measured by different sensors and pore water pressure and/or precipitation are usually used (Michoud et al., 2013). Various sensors can be set to monitor displacements, including extensometers (cable or laser) and crackmeters that measure the distances between two points, and total stations that are also used to provide distances and 3D positions using targets positioned on site. Moreover, GPSs are nowadays widely used, which can give the real 3D position of a point (Gili et al., 2000). All the above techniques usually provide data only at specific point locations; thus, several of them must often be set up in a network to monitor areal deformations. Inclinometers give deformations at depth along boreholes, providing essential data on the changes in depth of landslide behaviour (Blikra, 2008). For the last few years, ground-based interferometric radar (GB-InSAR) has been used for the most critical landslides (Casagli et al., 2010; Blikra, 2012; Rouyet et al., 2016). It provides a map of the distance changes, from the GB-InSAR to the landslide surface, at a millimeter scale and with a time resolution of a few minutes. Satellite InSAR images are also used to monitor long-term displacement trends, with results being strongly dependent on the type of treatment. In optimal cases, the time resolution is about 6 days, with millimeter precision and meter spatial resolution (Berger et al., 2012). Finally, as landslides react to water infiltration, many instruments are dedicated to monitor water: rain gauges, piezometers, thermometers, barometers, moisture content sensors and other meteorological data. Pore water pressure changes monitored with piezometers usually have a good correlation with slope movements (Michoud et al., 2013).
Behind the implementation of the monitoring part of EWSs is the understanding of the landslide mechanisms, that is, the identification of the main parameters controlling the movements of the landslide (Intrieri et al., 2012 and 2013). For this purpose, the design of a landslide conceptual model (LCM) is fundamental, since it will guide the type and the location of the sensors to install, and it is required to forecast landslide failure scenarios. The updating of an LCM must be continual during the whole life of an EWS. In addition, landslide failure may trigger other hazardous events in a cascade effect, such as tsunamis or dam breaks, which have to be considered in the EWS. The reasons why an EWS is implemented are either the identification of an unacceptable risk level or an increase in, or abnormal, landslide activity. Although the LMC implementation process provides reasons to fix appropriate sensors that will monitor the most significant failure initiation indicators, there are usually many practical constraints, such as topography, access, visibility and available resources. Ideally, the first data from a monitoring system are used to calibrate and fix alarm thresholds usually based on displacement velocities or accelerations, or pore water pressure or precipitations (Cloutier et al., 2015). This approach can be supported by failure forecast models, such as the Fukuzono method, or by more complex models (Crosta and Agliardi, 2003; Federico et al., 2012). The alarm thresholds will be used to trigger chains of actions that will involve different levels of people, depending on the alert level, from technicians and experts to officers and politicians who will be involved in the assessment of the abnormal situations and who will have to make decisions (Froese and Moreno, 2014). This starts from the initial check of the situation and the coherence of the movement detection of the sensors (to avoid false alarm), and it can end with an evacuation decision. It requires the monitoring system to be reliable and is therefore redundant in terms of sensors, communication and the stakeholders involved. Pre-defined crisis units must follow decision trees to propagate or stop the warning at each level. This also requires to constantly verify that the observed landslide behavior is still following the expected course, which also implies that the threshold and alarm levels can be reassessed by the crisis units.
The most important actions that can be prompted by EWS high-alert levels are evacuations and a rapid set-up of protection measures. They imply that all stakeholders, including the relevant population, must be prepared through education and training to implement the appropriate response.
In addition, the methods used to emit and communicate the emergency situation must be adapted to the local population culture. It must be stressed that all stages of implementation or operation must include feedback to the other stages. Frequent feedback and updates are a key point. They must also include the reappraisal of the indirect effects (cascade). A final problem relates to communication to the general population, which, to be effective, needs trust and training and must be an efficient means by which to communicate and emit warnings and actions within the noise of our ‘connected world’. It appears that only 38 % of the EWSs have more than one communication vector to inform the population (Michoud et al., 2013).”
…
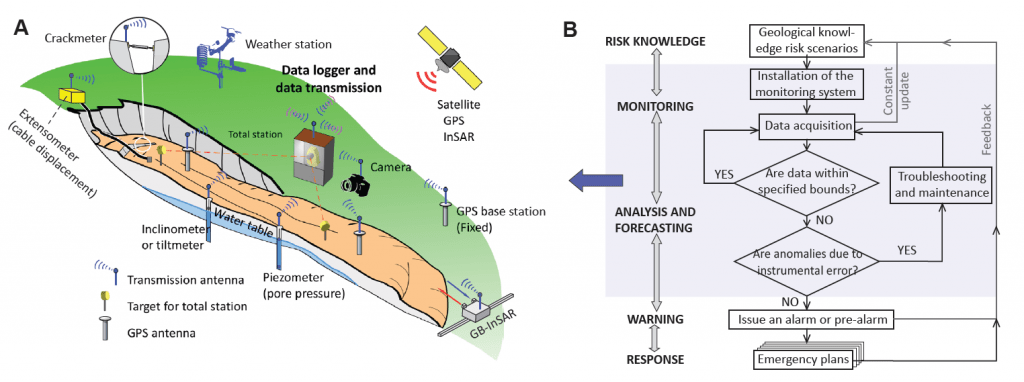